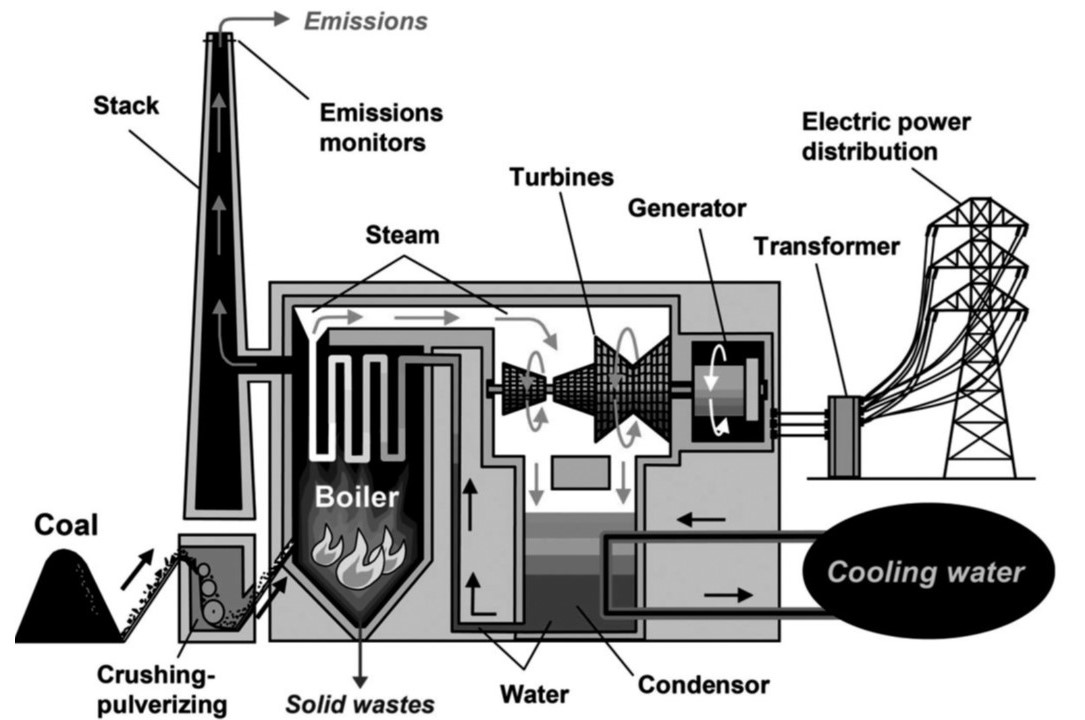
In my last column, I discussed the development of fossil fuels, which created the Industrial Revolution; the Industrial Revolution caused tremendous disruption, but overall helped free mankind from drudgery, and in fact, took away a main motivation for slavery, which had existed since ancient times.
The final major technological breakthrough, connecting the industrial revolution to the modern energy complex of today, was the electrification revolution that emerged in the late 1800’s. The fathers of the electric revolution were three men of great modern renown, and bitter rivals, Thomas Alva Edison, George Westinghouse (the businessman), and his scientific partner Nikola Tesla. All three men contributed greatly to scientific progress and invention, in the end the alternating current system of Westinghouse-Tesla would become the chosen form for producing mass electricity. Due to its inherent advantage of being able to be transmitted over long distances with lower transmission losses. This system persevered despite an aggressive propaganda campaign by Edison to demonize the safety of alternating current (AC) through the public electrocution of circus animals and convicted murderers.
After Edison solved the puzzle of the incandescent filament light bulb, he turned his attention to spreading the gospel of electricity and direct current. On January 12, 1882, Edison’s Electric Light Company began operation of the world’s first power plant in London. The coal fired power plant was known as Holburn-Viaduct Power Station. When operating the Power Plant produced about 95 kW of electrical power (125 HP) and had the capacity to illuminate 7,200 lamps across London. This first power plant produced Edison’s direct current electricity (DC) and the plant operated for only 4 years, before closing, following significant financial losses. Edison’s second adventure into power generation started operation the same year (September, 1882) at the Pearl Street Station in New York City and had a similar troubled operating history.
The first major Westinghouse-Tesla foyer in electrical power generation occurred in upstate New York at Niagara Falls, where a 5,000 HP alternating current hydroelectric power station was constructed between 1893 and 1896. First operation transmitted electrical power to the City of Buffalo about 30 kilometers away. The harnessing of the Niagara River continues to this day with capacity of the rebuilt (several times) and modernized hydroelectric plant currently standing at 2,500 MW (3.4 million HP). This coupled with the success of the Westinghouse-Tesla exhibit and the 1893 World Fair in Chicago, ended the “War of the Currents” in favor of the Westinghouse-Tesla alternating current system and provided a new blueprint for humanity to harness the power of combustion and electrification.
Now that we understand the broad strokes of the history that got us to this point, a key question remains: what is a power plant and how does it work? A power plant uses much the same principles as the Newcomen-Watt steam engine: a fuel is combusted or reacted in an engine, boiler or reactor.
How Power Plants Work
Using a coal-fired power plant as an example, coal is pulverized and then burned in a boiler. The boiler is encased in steel tubes that circulate water, which is then converted into high pressure steam (500-2000 psig). Water and/or steam is known as the “working fluid” and still to this day is the most common type of working fluid. High-pressure steam from the boiler is reduced (pressure lowered) by passing it through a turbine, which spins as it absorbs energy from the steam.
The turbine is connected to a spinning electrical generator. The electrical generator produces electricity through electromagnetic induction as a spinning copper induction coil is spun rapidly between the poles of a horseshoe-type magnet. The steam, after passing through the turbine, enters a heat-exchanging condenser, where it is cooled with circulating cooling water, condensed back from steam into water, pulling a vacuum in the process, increasing the pressure drop and power output of the turbine. In the final step the water is returned to the boiler where it is converted back into steam and the loop begins again (see Figure 1).

Pretty much every power plant follows the design shown in Figure 1. The coal-fired boiler can be replaced with an oil-fired boiler, but the back-end power-generating section remains unchanged. When combusting natural gas, the entire front end of the power plant is replaced with a gas turbine, where gas is directly combusted inside the turbine—effectively a large stationary jet engine. In a nuclear power plant, the entire boiler and front end are replaced with a nuclear reactor where controlled fission takes place, but in most cases water is still the working fluid, and the back end looks much like a conventional power plant. For the so-called renewable fuels, mainly wind and solar, the power plant is replaced up to the electrical transformer with either a field of spinning wind turbines or a field of photovoltaic power cells, directly converting the wind’s or sun’s energy into electricity.
Emissions
One key distinction that differentiates power plants in different parts of the world is how the emissions are handled. The fundamental chemistry equation governing combustion for methane (the simplest hydrocarbon) is:
CH4 + 2 O2 CO2 + 2 H2O
ΔH = – 891 kJ/mol
Perfect combustion of a fossil fuel or hydrocarbon produces three byproducts; carbon dioxide, water and heat (-891 kJ / mol). Unfortunately, perfect combustion does not exist in practical terms, as all hydrocarbons contain impurities that are absorbed in the formation process and interact with the combustion process. Coal and oil contain sulfur, nitrogen and heavy metals. Natural gas contains small amounts of sulfur and nitrogen that are typically removed during gas processing. These impurities and the physical reality of imperfect combustion produce detrimental emissions of sulfur oxide (SOx), nitrogen oxide (NOx), carbon monoxide (CO), particulate matter (PM) and vaporized heavy metals (especially from coal and oil), all of which have a deleterious effect on air quality and human health if breathed continuously and in high enough concentration. Due to these realities, many power plants (almost all in the West) come equipped with a second set of industrial pots and pans for the removal of harmful combustion emissions, excluding the carbon dioxide and water that come from perfect combustion.
Today, the Earth is home to approximately eight billion individuals whose aggregate energy consumption is the astronomically large number of 570 quadrillion BTUs or somewhere around 70 million BTUs per year per person. On a continuous basis, this calculates to a continual work usage rate for every person on the planet of ~2.0 HP or the antiquity equivalent of every person on the planet owning two horses, working around the clock.
It should be noted that energy usage isn’t distributed evenly across the planet. On one end of the scale are countries like the U.S., where the average energy consumption is 265 MMBTU per person per year or roughly four times the global average. Compare this to India with five times the population of the U.S,. where the average energy consumption per capita per year is a mere 25 MMBTU, one-tenth the per capita energy usage of the U.S.
Energy Scenarios for the Future
In 1900, the energy mix of the world was about 90 percent coal and 10 percent everything else. See page 19 for a discussion on each of the primary energy sources used in the world today; provided below is a graphical representation of our changing energy mix over time. It should also be observed that energy usage in the world as a whole is shifting from more concentrated sources such as wood and coal, to more diffuse sources of energy such as natural gas, hydrogen and direct electricity. As a fuel source moves from solid to gaseous and more dispersed states, the cost to produce, transport and store it increases geometrically, or at least it has for the past one hundred years.

Figure 2 is a projection by British Petroleum (BP), which shows how the energy mix of the planet would change with a rapid forced transition to low fossil fuels and high renewables usage. It represents one possible outcome of many. With all of these diverse energy sources available to mankind, the logical questions are: what is the best energy source, economically speaking, and what we should be investing in?
The real-world answer is: “it depends.” It depends on who you ask and what assumptions you make. The challenge, as with many comparisons, is factoring in the complex and patchwork system of regulations around the world that change the answer, depending on what country you reside in and the natural availability of resources in the region.
In general, the following variables all influence the cost of producing power, based on location and regulatory framework:
- Capital construction cost of the plant
- Delivered gate price of selected fuel
- Plant operating eosts
- Plant operating availability
- Electricity sales price
- Tax credits associated with different fuel types and plant configurations
- Additional costs to mitigate emissions or pay emissions tax
- Acceptable profit margin
- Financing terms
Each of these variables is highly specific to individual real-world projects and makes an apples-to-apples comparison of the cost of producing power highly subjective and a bit futile. Figure 3 provides a comparison of energy costs developed by the energy investment bank Lazard, which I believe is realistic. However, always remember that a good accountant or engineer, by tweaking the input assumptions, can change the result to suit the expectations of the intended audience.
Figure 3: Levelized Cost of Energy Comparison—Unsubsidized Analysis
Source: Lazard estimates.
Note: Here and throughout this presentation, unless otherwise indicated, the analysis assumes 60% debt at 8% interest rate and 40% equity at 12% cost. Please see page titled “Levelized Cost of Energy Comparison—Sensitivity to Cost of Capital” for cost of capital Sensitivities. These results are not intended to represent any particular geography. Please see page titled “Solar PV versus Gas Peaking and Win versus CCGT—Global Markets” for regional sensitivities to selected technologies.
- Unless otherwise indicated herein, the low case represents a single-axis tracking system and the high case represents a fixed-tilt system.
- Represents the estimated implied midpoint of the LCOE of offshore wind, assuming a capital cost range of approximately $2,600 – $3,675 kW.
- The fuel cost assumption for Lazard’s global, unsubsidized analysis for gas-fired generation resources is $3.45 / MMBTU.
- Unless otherwise indicated, the analysis herein does not reflect decommissioning costs, ongoing maintenance-related capital expenditures or the economic impacts of federal loan guarantees or other subsidies.
- Represents the midpoint of the marginal cost of operating fully depreciated gas combined cycle, coal and nuclear facilities, inclusive of decommissioning costs for nuclear facilities. Analysis assumes that the salvage value for a decommissioned gas combined cycle or coal asset is equivalent to its decommissioning and site restoration costs. Inputs are derived from a benchmark of operating gas combined cycle, coal and nuclear assets across the U.S. Capacity factors, fuel, variable and fixed operating expenses are based on upper- and lower-quartile estimates derived from Lazard’s research. Please see page titled “Levelized Cost of Energy Comparison—Renewable Energy versus Marginal Cost of Selected Existing Conventional Generation”for additional details.
- High end incorporates 90% carbon capture and storage. Does not include cost of transportation and storage.
- Represents the LCOE of the observed high case gas combined cycle inputs using a 20% blend of “Blue”hydrogen (i.e., hydrogen produced from a steam-methane reformer, using natural gas as a feedstock, and sequestering the resulting CO2 in a nearby saline aquifer). No plant modifications are assumed beyond a 2% adjustment to the plant’s heat rate. The corresponding fuel cost is $5.20 / MMBTU.
- Represents the LDOE of the observed high case gas combined cycle inputs using a 20% blend of “Green” hydrogen (i.e., hydrogen produced from an electrolyzer powered by a mix of wind and solar generation and stored in a nearby salt cavern). No plant modifications are assumed beyond a 2% adjustment to the plant’s heat rate. The corresponding fuel cost is $10.05 / MMBTU.
Figure 3 is just one example of a comparison that accurately frames the comparative levelized cost of producing energy and incorporates the various tax credit subsidies that are available for renewable power generation but not fossil fuels. Notice the number of explanatory footnotes and assumptions. As an example of where one assumption can change the entire analysis, pay close attention to Note 3, where they state that the assumed price of natural gas for the analysis is $3.45 / MMBTU. Compare that to the average benchmark price of natural gas (Henry Hub Basis) in the U.S. for the past ten years, which has averaged $2.56 / MMBTU, or 25 percent lower than what was assumed as the natural gas fuel cost in this particular analysis.
Over the past two hundred years, humanity has demonstrated a full mastery of Prometheus’ gift of fire and scaled the use of energy from local combustion to gargantuan levels. We harness this thermal energy to produce our power needs and displace incremental human labor—avoiding deforestation in the process. It is not a coincidence that during this period as a society we have ended the immoral and barbaric practice of human bondage for agriculture and construction and replaced it with cheaper mechanical labor.
But has our mastery of combustion tipped the scales too far, where the rerelease of hundreds of millions of years of concentrated sunlight back into the atmosphere is leading us to a day of reckoning? Nobody knows the answer, but I can assure you that vast swaths of the political elite, scientific community and the consulting industrial complex are squarely focused on providing that answer—or at least providing an answer that best suits their current agendas.
Wind and Solar Energy Question
I’ll end this discussion with a practical question. At current population levels and average current energy consumption, could we replace all the fossil fuel usage in the world with wind and solar and how much land would that require? The answer is about thirty million square miles, depending on the mix of wind and solar, or roughly 50 percent of all land currently above sea level—this is the amount of land that we would need for solar and wind farms to maintain the current global energy standard of living for eight billion inhabitants without using fossil fuels or nuclear power generation.
Policymakers are facing the mother of all Malthusian energy crunches. Personally, I expect pragmatism to win the day and take hold of this monumental challenge for the planet, where twenty years from now the energy mix will look like 25 percent natural gas, 25 percent oil and petroleum, 10 percent coal, 30 percent renewables and nuclear and 10 percent emerging exotic forms of energy.
Sidebars
Energy and Power Unity of Measure
The primary units of energy and power measurement used in this article include the following:
- BRITISH THERMAL UNIT (BTU): The amount of heat energy required to increase one pound of water one degree Fahrenheit. MMBTU is one million BTU
- HORSEPOWER: A measure of useful energy produced/consumed over time (defined as work or power) produced by a standard horse, 1 BTU per second is equal to roughly 0.71 HP.
- WATT OR KILOWATT (KW): The metric measurement for power, where 1 HP is equal to 745.7 watts or 0.746 kilowatts or .000746 megawatts.
- KILOWATT-HOUR: The standard measure of power production over time. Both horsepower and the watt are instantaneous units of measure, typically a heat value (BTU or Joule) per second. A KW-Hour represents 1 KW of power generated continuously for 3600 seconds or 1 hour.
Energy Crisis in Europe
There is an energy crisis brewing in Europe due to a combination of low wind velocity, decreased natural gas deliveries from Russia, and skyrocketing carbon credit prices, which have resulted in a tripling of European electricity prices over the last six weeks, from roughly $100 GBP / MWh to over $300 GBP / MWh.
Recent ripples in the European energy markets provide an ominous foreshadowing of dark things to come. Most of the world has embraced the new political religion of the green eco-warrior, valuing all things “renewable” and shunning the apparent dirtiness of hydrocarbons. This has resulted in a large-scale capital redistribution away from coal, oil and even natural gas toward wind turbines and solar photovoltaic arrays. Nowhere has the push been stronger than in Europe, specifically for electricity generation.
In fact, 2020 proved to be a banner year for Europe’s decarbonization push, where electricity from renewables exceeded electricity produced from traditional fossil fuels, specifically coal. One of the triumphant headlines, seen over the course of 2020 and 2021, was that there were months across the year where Britain produced no electricity from coal. As the champagne corks pop, however, we need to remember that old saying, “Don’t count your chickens before they hatch.” Although there is no technical issue with producing electricity from solar and wind, both come with two explicit downsides that policymakers have overlooked or ignored: intermittency of supply and the large amounts of real estate necessary for industrial-scale production.
Over the past two months, the wind hasn’t blown as hard as Europe was expecting. One current estimate puts United Kingdom wind capacity as operating at only 10-20 percent of capacity for much of the past year. When the wind stops blowing, the back-up plan is to use natural gas-fired “peaking” plants to offset the shortfall. The majority of Europe’s natural gas supply comes from three sources: pipeline gas from North Africa, imported liquified natural gas (LNG) from the Middle East and the United States, and Russian pipeline gas, Russia being by far the largest source by volume and energy content. Whether through operational issues, financial impairments or outright political motivations, the supply of gas from Russia to Europe has dwindled.
Unbeknownst to much of the outside world, one of Russia’s strategic energy thrusts over the past ten years has been to diversify the customer base for their natural gas production away from Europe, their sole external customer base since the 1970s. They have done this primarily through building pipelines and LNG facilities in the East to give them the option to sell their gas bidirectionally, effectively pitting Europe against China, Japan and Korea for access to Russian energy molecules.
With wind’s recent non-performance and a new Eastern market for Russian gas, Europe is suddenly finding itself short of energy. The only immediate solutions available to Europe are either to shut down large industrial energy users (factories and manufacturing) or fire up old coal-fired power plants on the verge of mothballing. The problem with the latter option is that within Europe’s integrated carbon credit and trading system, coal-fired power generation requires the purchase of a large volume of increasingly expensive carbon credits to legally support operation.
The end result of all of this is both intermittent power supply and much higher cost. So who ends up footing the bill for these shortsighted policy decisions? The consumer does! This emerging issue has the potential to grow into a full-blown energy crisis as winter looms. We can also expect an impact on U.S. gas and electricity markets through the interconnectivity provided by U.S. LNG exports.
Primary Energy Sources and Usage
FOSSIL FUELS
COAL is used primarily to produce heat from direct burning and electricity in power plants, and represents roughly one-third of primary energy usage. The second main use of coal is as a direct input into the production of steel. Thus the world of coal is split between thermal and metallurgical use along with a couple of niche applications, such as converting coal into a liquid fuel through the Fischer-Tropsch process, also known as coal-to-liquids (CTL).
PETROLEUM includes crude oil and the products made in a refinery, including gasoline, diesel and jet fuel. Petroleum is primarily used as a transportation fuel; about 85 percent of transportation energy is petroleum-derived. Petroleum liquids have superior energy density (energy per unit of volume) and are easily transported long distances, making them an ideal transportation fuel. Outside of transportation, the other dominant use of petroleum is for producing petrochemicals—close to 10 percent of every barrel pumped from the ground is converted into petrochemical products. Outside of a few specific places in the world, petroleum is no longer heavily used in power generation, and its use as a heating and cooking fuel has largely been displaced by natural gas and light hydrocarbons such as propane and butane. Today, oil consumption stands at about 100 million barrels per day, although the world did experience a 15 percent drop in petroleum demand due to the global pandemic, this has quickly rebounded in the past year.
NATURAL GAS has similar applicability and uses as coal. Natural gas is mostly methane, creates less carbon dioxide emissions per unit of energy produced and has far less harmful impurities than coal or oil. For these reasons and because of its newfound abundance due to hydraulic fracturing, natural gas has become the de facto choice for replacing coal in electricity generation. The challenges of gas remain diffusion and portability. Gas molecules are tiny and have a tendency to leak, and uncombusted methane is a more potent greenhouse gas than carbon dioxide. Natural gas is very difficult to transport outside of pipelines and compressors. In order to make natural gas suitable for seaborne international trade, it is converted into a liquid (liquified natural gas, LNG) by chilling it to -300 degrees F and moving it in specially designed insulated tankers. The entire process is capital- and energy-intensive but does exist; natural gas is growing rapidly as a traded commodity.
WOOD AND ANIMAL WASTE are still part of the energy mix, but minor and declining fast. Across large swaths of Africa and India, wood and dung are still used as the primary source of energy for heating and cooking. There are substantial efforts globally, in India in particular, to eliminate this practice and replace wood with less polluting liquified petroleum gas (propane and butane). There is also a collection of power plants in the world (mostly in Europe) where wood is converted into briquets called torrefied wood pellets and used as a power-generating fuel instead of coal.
RENEWABLES
HYDROELECTRIC involves damming a river and installing a turbine-based power plant at the base of the dam; electricity is generated through the gravity-driven descent of water from the dam. There are some truly enormous hydroelectric facilities in the world such as the Hoover Dam in the U.S. (2,080 MW or 2.8 million HP) and the Three Gorges Dam in China (22,500 MW or 30.2 million HP). Hydroelectric is technically a renewable energy source; however, this does not mean that it has no environmental consequences. The damming of huge rivers to create lakes on the upstream side of the dam is massively disrupting to the local ecosystem and can become an activist focal point during droughts and other water scarcity events. Consider this: construction of the Three Gorges Dam in China involved the forced relocation of 1.5 million citizens. Hydroelectric is certainly viable going forward, but there are only so many suitable rivers in the world and many rivers cross multiple jurisdictions, leading to an inevitable conflict around who owns and controls the water.
WIND POWER, the modern-day version of the windmill, is generated from a field of wind turbines, spinning as the wind blows to produce electricity. Today, the standard wind turbine is two hundred fifty feet high, fifty feet thick and contains three massive blades producing on average 1.5 MW or 2,000 HP per turbine. The challenge with wind is intermittency (matching wind velocity with changing electricity demand throughout the day) and storage. Wind turbines directly produce electricity, which is very expensive and hard to store. Battery storage of electricity is a technology receiving huge amounts of interest and funding to address this challenge; large-scale storage of electrons is still very much in its infancy. The other problem with wind is physical space. Wind turbines take up a lot of space. A one thousand MW gas-fired power plant typically has a physical footprint of one thousand acres. The same amount of energy produced from seven hundred wind turbines would require a physical footprint of almost two hundred thousand acres.
SOLAR is similar to a wind farm in terms of its pollution footprint and also suffers from many of the same constraints as wind. A solar farm uses solar panels (polysilicon photovoltaic grids) to absorb energy from the sun and convert it directly into electricity. The problem with the sun is it is far away, the energy that it projects is diffuse by the time it reaches Earth and there is intermittency with nighttime and cloud cover. The solar constant, the amount of power the sun deposits per unit of surface area, is about 1 kW per square meter under direct sunlight exposure. A one-thousand MW solar farm requires almost forty thousand acres. Despite these constraints, the modern appeal for widely available solar generation remains strong and the costs have decreased considerably in the past decade, largely due to massive R&D and industrial factory development in China and Japan.
GEOTHERMAL AND TIDAL are both very much niches and will likely never comprise a significant portion of the energy mix. Geothermal works like a conventional power plant, spinning a turbine using geothermal steam from the depths of the earth. It has some application but is very geologically attached to places like Iceland and Northern California. Tidal uses the changing elevation of tidal flows to power a small turbine and has been experimented with on islands for many years; however, the five-to ten-foot change in tidal elevation is not enough to generate substantial amounts of energy; in my opinion tidal will forever be the domain of PhD student research projects.
EXOTICS
NUCLEAR FISSION is an utterly viable solution for large-scale, small-footprint, emission-free power generation. If fully exploited, there is enough nuclear fuel on the planet to power the Earth and its people for a millennium. Since the 1970s, nuclear has been the preferred path for developing countries with large electrified populations and very little natural endowment of fossil fuel resources (France, Japan, Korea, China, etc.), and a substantial amount of generating capacity was built. No other fuel or power-generating option better represents the dichotomy of Prometheus’ Gift and Pandora’s Curse than nuclear. The challenge with nuclear remains safety and managing radioactivity. As major disasters at Chernobyl and Fukushima have shown us, this is no small task. Nuclear remains viable, but I doubt very little new capacity will be added due to the overwhelming and very real concerns of safety.
NUCLEAR FUSION is not currently technically viable. Is it the ultimate dream or folly of humans to attempt to replicate the process that powers the sun? Many practical and technical challenges remain with fusion and a large volume of R&D breakthroughs would be required to make this emerging technology commercially viable. The current best chance for producing meaningful power from fusion is the thirty-five-nation ITER project under construction in France. If it works, it could produce 500 MW of power, but this is not expected until much later this decade. Assuming a huge amount of scientific progress, fusion might be a viable power-producing technology in fifty years.
HYDROGEN – Stop me if you’ve heard this one before: hydrogen is the most abundant element in the universe, so why don’t we transition to a hydrogen economy? This is a true but deeply misleading statement. Yes, hydrogen is the most abundant element in the universe and the combustion of hydrogen does produce heat and energy without the inconvenience of producing carbon dioxide. Hydrogen combusts to water and water alone, with no carbon in the equation. The problem with hydrogen is that it does not occur naturally as an isolated element in material quantities. About 99.5 percent of the hydrogen available on the planet today is produced from reforming natural gas, where methane is converted to hydrogen and the associated carbon molecule is expelled into the atmosphere in the form of carbon dioxide. At present, hydrogen is sexy and touted as the “next big thing.” The current thinking is to use hydrogen as a sort of battery in combination with solar and wind. Surplus electricity from solar and wind is fed to a hydrogen electrolyzer, where electricity separates water into hydrogen and oxygen. The hydrogen is then piped or compressed and used in further power generation or to power vehicle propulsion through a fuel cell. Unfortunately, all this converting back and forth is thermodynamically inefficient; hydrogen also remains one of the most difficult molecules to store and transport safely.
Where the Power Plants Are
For further information on the energy mix and balance of the world, your country or your local region, there are a couple of excellent publicly available resources that can be utilized. For the macroscopic energy picture, I would recommend BP Energy’s statistical handbook: bp.com/en/global/corporate/energy-economics/statistical-review-ofworld-energy.html.
For the U.S., I suggest the Energy Information Administration’s (EIA’s) energy portal, which allows you to see the size, location and generating mix of every power plant in every region of the U.S.: eia.gov/state/maps.php.
This article appeared in Wise Traditions in Food, Farming and the Healing Arts, the quarterly journal of the Weston A. Price Foundation, Fall 2021
🖨️ Print post
Leave a Reply